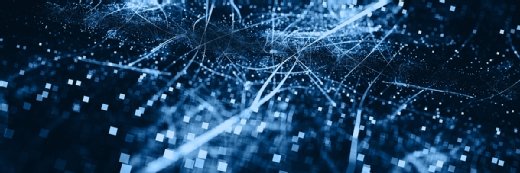
Getty Images
An introduction to quantum networks and how they work
Quantum networks hold potential for faster and more secure communication. But true quantum networks are still experimental and require more engineering and scientific research.
Quantum networking connects systems and transmits data among them using quantum phenomena. This concept differs from classical network technology based on photon or electron transmission.
Currently, commercial quantum networking mainly refers to quantum-secured networks. These data networks use conventional methods, such as TCP/IP over fiber and copper, for data transmission, while using a parallel quantum network to generate and share encryption keys that secure the data stream.
Teams in many places are working toward true quantum networking that uses quantum channels to transmit entire data streams.
Many research labs and technology incubators have instantiated quantum-secured networks, including MIT Lincoln Laboratory, Caltech Quantum Network and Los Alamos National Laboratory. Commercial implementations are under development in New York, California and Tennessee, among other places.
Underlying principles of quantum theory
Several aspects of quantum behavior make quantum networking possible. Here are some important quantum principles.
Entanglement
Quantum entanglement is the phenomenon when two quantum systems become linked and share fundamental behaviors in a unified quantum state. For example, two entangled particles share properties such as spin, polarization and conservation. Any change in the basic quantum characteristics of one particle requires an immediate and predictable change in the other particle, regardless of the distance between them.
Take, for example, two entangled photons spinning in a singlet state. The conservation principle states that the total spin of an entangled group must be zero. The two photons have opposite spin orientations and maintain a total spin momentum of zero. But, if one photon starts spinning from down to up, the other switches from up to down to keep the total spin momentum at zero.
Quantum bits (qubits) entangled with each other can transmit data. If a qubit at one end of the network changes state, the entangled qubit at the other end of the network instantly reflects the correlated changes. Bits of data are communicated by observing these changes.
Coherence
The quantum state of a system, such as two entangled qubits, needs to be maintained for data to flow. If the state degrades, the system loses coherence, which refers to the synchronization and correlated behaviors of the quantum system.
Various factors can disrupt coherence, such as electromagnetic fluctuations, temperature changes and quantum measurement. For example, a qubit that becomes entangled with surrounding matter causes decoherence with its entangled qubit.
No-cloning
Quantum laws dictate that observation of a system changes its state. As a result, qubits cannot be copied directly. So, for example, the no-cloning principle prohibits exact backup copies, as that process would disturb the original state.
How quantum networking works
The end nodes on a quantum network house quantum processors that generate and receive qubits. They might also have some quantum memory capacity.
Below are some methods quantum networking uses to connect end nodes:
- Direct connection. One example of this method is sending entangled photon beams in free space to connect endpoints without using a physical medium.
- Indirect connection. This method commonly uses optical fibers and specialized optical switches to connect end nodes. Entangled photons travel through the fibers, which maintains the quantum states of the qubits carrying data.
For longer distances, specialized quantum repeaters move traffic along multiple, shorter hops. Quantum repeaters can move traffic either as trusted or untrusted participants in the communication chain.
Trusted repeaters enable end-to-end communications via a chain of quantum key distribution (QKD) events. These events give the end nodes a securely generated and transmitted encryption key that is also known to one of the intervening repeaters.
Untrusted repeaters are purely quantum repeaters. These repeaters perform a quantum operation -- called a Bell measurement -- on pairs of qubits to entangle them, so changes in one are reflected in the other. A transmitting end node creates a pair of entangled qubits, holding one and sending the other through the repeater network.
A repeater entangles the incoming qubit with one of a pair it has generated internally. Any changes in the state of the qubit from the transmitting end node are reflected in the state of the qubit from the repeater. The second repeater qubit travels to the next repeater in line, and the process repeats and extends the entanglement until the qubit reaches the receiving end node. At that point, changes in the qubit held on a transmitting node result in changes in the sent qubit on the receiving node, enabling information to flow.
Use cases of quantum networking
Once a quantum transmission link is established, the communications channel is intrinsically secure. It can't be intercepted or copied without corrupting the data. Quantum networking is, therefore, attractive in any use case requiring completely secure networking -- within a data center, across a campus, on a metro area network or on a WAN.
Another main use case is creating quantum-linked clusters of quantum computers, independent of the need for security on such links.
A weaker form of quantum-secured conventional networks -- QKD -- is commercially available. The data stream is subject to interception and copying, as quantum mechanics were used only to transmit the encryption keys. But, if the system transmits long encryption keys that are used for symmetric encryption -- as opposed to public key encryption -- then the transmitted data should be resistant to decryption even by quantum computers.
Challenges of quantum networking
True quantum networks are still experimental. Quantum links of more than 10 kilometers have been established using fiber optic cables, and links have even been established between ground stations and satellites. But much work remains in increasing the reliability, throughput and efficiency of the technology, while also reducing the costs. Efforts to commercialize the science are well under way, but it will take time for commercially viable quantum networks to become available.
Scientific and engineering work is required to make quantum networking practical. Some major challenges include improvements in error correction, network coherence and qubit generation. With QKD a reality, however, it is clear true quantum network is coming.